Table of Contents
- What Is CRISPR-Cas9 and How Does It Work?
- Why AAV Is the Leading Vector for CRISPR Gene Delivery
- Key Tools and Technologies for AAV-CRISPR Delivery
- In Vivo Gene Editing with AAV-CRISPR: Methods and Real-World Examples
- Current Challenges and Future Directions of CRISPR-AAV Therapies
- PackGene’s AAV and Off-the-Shelf CRISPR/Cas9 rAAV Solutions
- References
CRISPR-Cas9 gene editing has transformed biomedical research, offering unprecedented precision in modifying DNA. However, delivering CRISPR components efficiently and safely into target cells remains a major hurdle. Adeno-associated viruses (AAVs) have emerged as a leading delivery vehicle due to their safety, tissue specificity, and long-term gene expression.
What Is CRISPR-Cas9 and How Does It Work?
CRISPR-Cas9 is a groundbreaking gene-editing tool originally derived from a natural immune defense system found in bacteria. It has revolutionised genetic engineering due to its precision, efficiency, and versatility.
CRISPR-Cas9 is a two-component system:
- Cas9 Nuclease: This is the molecular “scissors” that cuts double-stranded DNA at a specific site in the genome. Once the DNA is cleaved, the cell’s natural repair mechanisms are activated.These repair pathways, mainly non-homologous end joining (NHEJ) or homology-directed repair (HDR), can be harnessed to disrupt genes or introduce precise genetic changes.
- Guide RNA (gRNA): This is a short synthetic RNA molecule that directs Cas9 to the precise target sequence. It does so by forming complementary base pairs with the DNA, ensuring the cut is made at the correct location. In addition to matching the target DNA, successful binding and cutting also require the presence of a nearby PAM (Protospacer Adjacent Motif) sequence — for example, 5′-NGG-3′ for SpCas9. The PAM is essential for Cas9 to recognise and cleave the DNA.
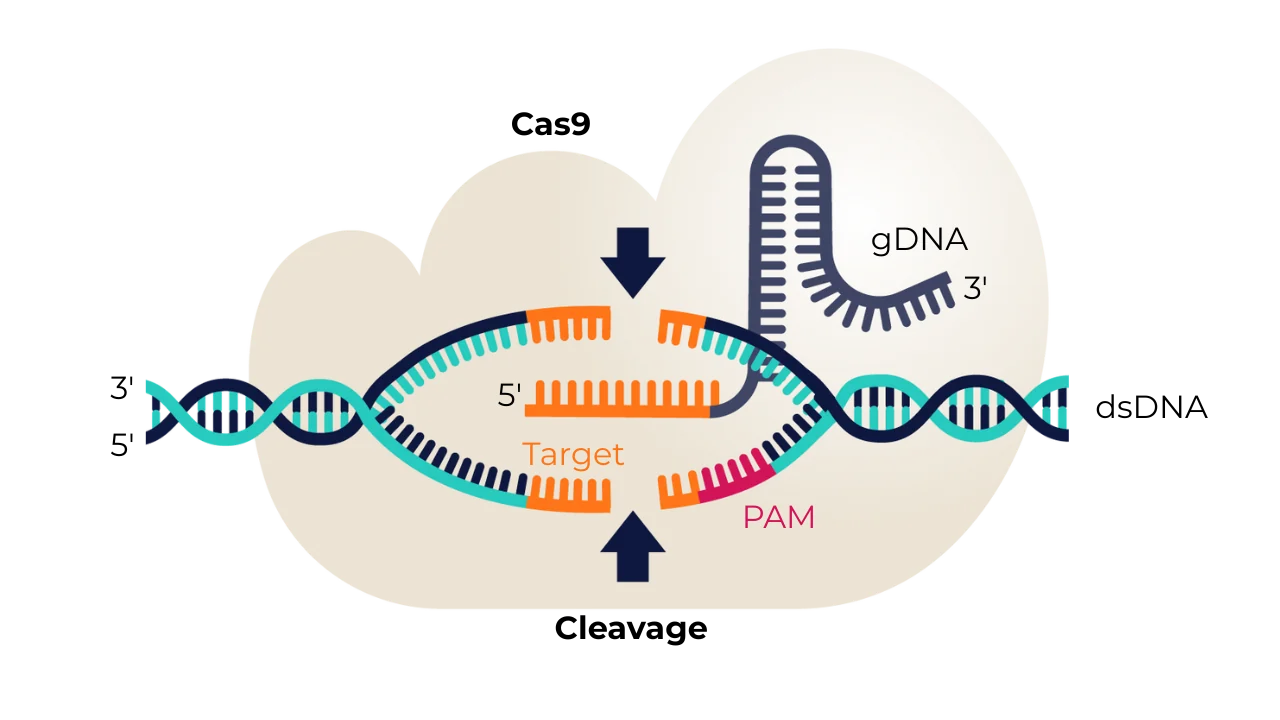
What Happens After the DNA Is Cut?
After Cas9 cleaves the DNA, the cell detects the break as damage and rapidly activates its natural repair systems. The choice of repair pathway determines whether the DNA is simply patched up with errors or precisely rewritten, which is critical for the outcome of genome editing.
1. Non-Homologous End Joining (NHEJ)
- This is the cell’s quick-fix method.
- It directly ligates the broken DNA ends together but is error-prone, often resulting in small insertions or deletions (indels).
- Use case: These random changes can disrupt gene function — ideal for gene knockout experiments.
2. Homology-Directed Repair (HDR)
- This is a more precise but less efficient pathway.
- The cell uses a repair template (which can be supplied by researchers) to insert or correct specific DNA sequences at the break site.
- Use case: Perfect for gene correction or knock-in strategies, such as introducing a therapeutic gene variant.
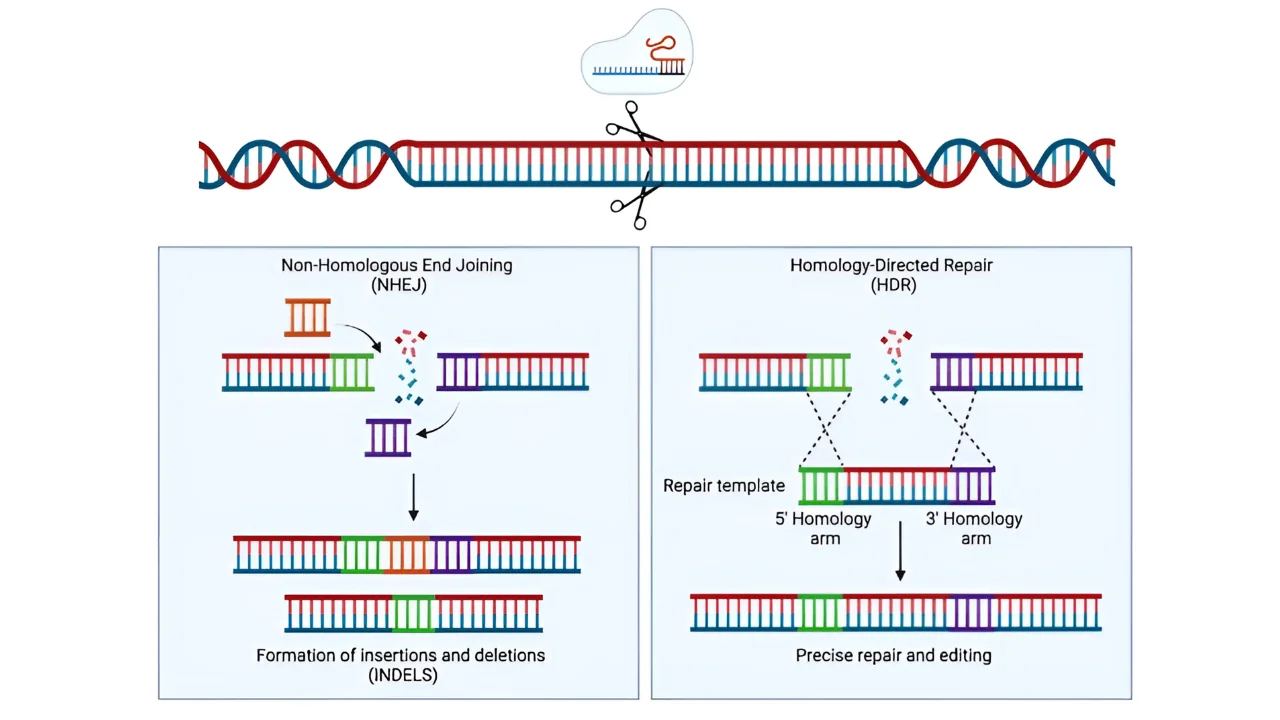
Why AAV Is the Leading Vector for CRISPR Gene Delivery
Efficient delivery of CRISPR components into cells is crucial for successful genome editing. Among various delivery methods, AAVs have emerged as a popular choice.
AAVs are ideal for CRISPR delivery because they:
- Infect both dividing and non-dividing cells:
AAVs can efficiently enter a wide variety of cell types, including non-dividing cells such as neurons, muscle fibers, and hepatocytes (liver cells). This broad tropism is crucial for targeting tissues that are otherwise difficult to edit.
- Have low immunogenicity compared to adenoviruses or lentiviruses:
AAVs are generally well-tolerated by the immune system, leading to lower risks of severe immune responses. This makes them safer for in vivo applications, especially for long-term or repeated treatments.
- Provide long-term expression:
Once delivered, AAV genomes persist mainly as episomes (non-integrated DNA) in the nucleus, allowing for stable, sustained expression of the CRISPR components over months or even years — important for diseases requiring ongoing gene modification.
- Offer a range of serotypes with different tissue targeting (tropism):
Different AAV serotypes naturally prefer different tissues (e.g., AAV9 for heart and muscle, AAV8 for liver, AAV2 for retina). Researchers can select or engineer specific serotypes to enhance delivery efficiency and minimise off-target effects.
Packaging CRISPR into AAVs
While AAVs are highly effective vehicles for CRISPR delivery, they are limited by a relatively small cargo capacity of approximately 4.7 kilobases (kb). This makes it challenging to fit both the Cas9 protein and the sgRNA into a single AAV. For example, SpCas9 (from Streptococcus pyogenes), the first widely used Cas9 enzyme, is relatively large (~4.2 kb), leaving little room for essential regulatory elements and guide RNAs. A smaller and more AAV-compatible Cas9 enzyme, SaCas9 (from Staphylococcus aureus), was later identified as a promising alternative, offering the advantage of easier packaging into AAV vectors due to its smaller size (~3.2 kb).
To overcome the size limitation of AAVs, several innovative strategies and approaches have been developed:
Delivery Strategies and Innovations to Overcome Size Limits
- Single AAV System:
This approach involves packaging both the Cas9 protein (such as SaCas9) and the gRNA into a single AAV vector. It’s a straightforward and high-efficiency method, ideal for small genome edits. However, it is limited by the AAV cargo size, making it suitable only for smaller Cas9 variants like SaCas9. This system works well for simple gene knockout experiments or targeted mutations, where smaller edits are sufficient. - Mini-Cas Proteins:
One of the most promising innovations is the use of miniature Cas proteins, such as Cas12f (~1.5 kb). These smaller Cas proteins have a significantly reduced size compared to traditional Cas9 enzymes, allowing them to be easily packaged into a single AAV vector without the need for splitting. Mini-Cas proteins maintain genome-editing functionality while overcoming the size limitations of AAV packaging, making them ideal for a wider range of applications, including those that require small but precise genome modifications. - Split Cas9:
This strategy involves splitting the Cas9 protein (e.g. SpCas9) into two parts, each packaged into separate AAV vectors. These vectors are delivered to the target cells simultaneously, where the Cas9 components reassemble and perform genome editing. This system enables the use of larger Cas9 proteins, such as SpCas9, which can be used for more complex edits. However, this split AAV system has some drawbacks, including lower efficiency (as both vectors must co-infect the same cell) and increased complexity in coordinating the delivery. - Dual AAVs:
Another solution to the packaging challenge is the use of dual AAV systems, where the Cas9 protein and its corresponding gRNA are packaged into separate AAV vectors. Similar to the split system, these vectors are co-delivered to the target cells, where the components assemble and perform the desired gene editing. This approach effectively overcomes the AAV’s cargo size limitation by distributing the components across multiple vectors. It also allows for the use of larger Cas9 proteins while maintaining the capacity to deliver the necessary regulatory elements. Likewise to the split system, this method requires careful coordination to ensure that both AAV vectors infect the same cell efficiently.
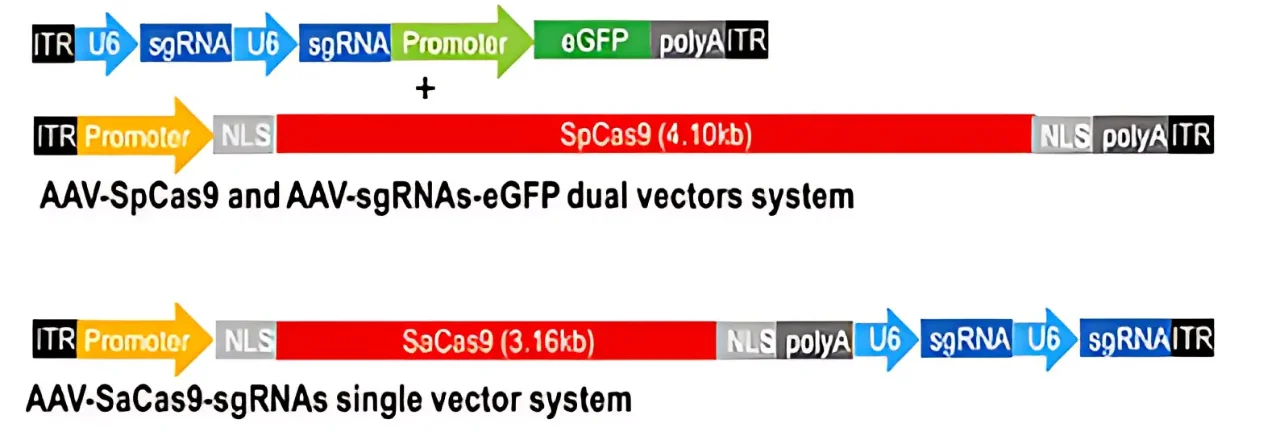
- Smaller Promoters
Using smaller promoters to drive the expression of Cas9 and sgRNA can free up space in the AAV payload, allowing for the inclusion of other necessary elements. For example, engineered promoters can be used to achieve high expression levels while maintaining a compact size.
Key Tools and Technologies for AAV-CRISPR Delivery
AAVs are a widely used choice for CRISPR delivery due to their ability to efficiently transduce a broad range of cell types while maintaining low immunogenicity. However, optimising AAV delivery requires several key tools and strategies to ensure targeted and effective genome editing. These strategies focus on enhancing tissue-specific expression, gRNA design, repair template delivery, and temporal control over Cas9 activity.
- Promoters for Tissue-Specific Expression: Promoters are crucial for controlling where and when the CRISPR components, such as Cas9 and gRNA, are expressed within the target organism. Different promoters allow for tissue-specific expression, minimising off-target effects and enhancing gene editing precision. Common promoter types include:
- Ubiquitous Promoters: Examples such as CAG and EF1α enable broad expression across many cell types, making them suitable for general gene editing applications.
- Liver-Specific Promoters: TBG and ApoE are used to target the liver specifically, which is essential for liver-based gene therapies and for reducing unwanted expression in other tissues.
- Neuronal Promoters: Synapsin and CaMKIIα promoters drive expression specifically in neural tissues, enabling targeted gene editing in the brain and nervous system.
- Muscle-Specific Promoters: Promoters like MHCK7 and Desmin are designed to target muscle cells, which is important for applications related to muscle disorders.
- gRNA Design & Expression: The gRNA directs the Cas9 protein to the correct genomic location, so its design is crucial for the precision of CRISPR-mediated genome editing. Key aspects of gRNA expression include:
- Pol III Promoters (U6 or H1): These promoters are used to drive the expression of gRNAs, ensuring their transcription as small RNAs by RNA polymerase III. The U6 and H1 promoters are commonly used in CRISPR experiments to maintain high gRNA expression levels for efficient genome editing.
- Multiplexed gRNAs: In cases where multiple genes need to be edited simultaneously, multiplexed gRNAs are employed. If space within the AAV vector allows, multiple gRNAs can be packaged into a single AAV, enabling the editing of multiple targets in one delivery. This is particularly useful for complex gene-editing tasks, such as genome-wide screenings or multi-gene therapeutic applications.
- Repair Templates for Homology-Directed Repair (HDR): HDR is the preferred pathway for precise genetic edits like point mutations or larger insertions. To facilitate HDR, a donor DNA template is co-delivered with the CRISPR components, allowing the desired genetic changes to be incorporated into the genome. Key points include:
- Co-Delivery of Donor Templates: The donor template, designed to match the genetic change, is either packaged in the same AAV or in a separate vector. This template facilitates the integration of the desired edit via the HDR pathway.
- Dual-AAV HDR Strategies: For larger genetic edits, dual-AAV systems are often necessary. These strategies are optimized to improve HDR efficiency, especially when dealing with larger gene constructs or complex repair templates that exceed the cargo capacity of a single AAV.
- Regulatable Systems: Controlling the expression of CRISPR components after delivery can significantly improve safety and efficacy by minimizing off-target effects and reducing unwanted immune responses. Some of the key regulatable systems include:
- Drug-Inducible Cas9 Systems: These systems allow Cas9 expression to be controlled by the administration of specific drugs, such as tetracycline or doxycycline. This enables temporal regulation, ensuring that Cas9 is active only when needed, which is critical for applications requiring precise control over gene editing activity.
- Self-Inactivating Systems: These systems are designed to limit the duration of Cas9 expression, reducing the risk of off-target effects. The Cas9 activity is transient, and once the editing process is complete, the system “self-inactivates,” ensuring that the gene-editing process doesn’t continue beyond the desired time frame, enhancing the overall safety of the approach.
Together, these tools and strategies provide critical enhancements to AAV-CRISPR delivery, ensuring that the gene-editing process is both effective and safe for a variety of therapeutic applications.
Category | Key Tools/Elements | Callouts / Benefits |
---|---|---|
Promoters | • Ubiquitous: CAG, EF1α • Liver-specific: TBG, ApoE • Neuronal: Synapsin, CaMKIIα • Muscle-specific: MHCK7, Desmin | Tissue-specific expression; reduces off-target activity |
gRNA Design | • U6, H1 promoters (Pol III) • Multiplexed gRNAs | Efficient targeting; supports complex genome edits |
Repair Templates | • Donor DNA co-delivery • Dual-AAV HDR strategy | Precise edits (e.g., point mutations or insertions) |
Regulatable Systems | • Drug-inducible Cas9 (e.g. Tet/Dox) • Self-inactivating Cas9 systems | Temporal control; reduces off-target and immune risk |
In Vivo Gene Editing with AAV-CRISPR: Methods and Real-World Examples
In vivo genome editing involves directly delivering CRISPR components into living organisms, enabling systemic or tissue-specific modifications without ex vivo cell manipulation. AAVs are particularly well-suited for this approach due to their ability to target specific tissues efficiently.
- Direct Therapeutic Application: In vivo editing eliminates the need for cell extraction and reinfusion, simplifying the process and reducing associated risks.
- Broad Tissue Targeting: AAVs enable the delivery of CRISPR components to multiple organs simultaneously (e.g., liver, muscle, CNS), offering a wide range of therapeutic possibilities.
- Permanent or Long-Lasting Effects: AAVs provide sustained expression, ensuring long-term therapeutic benefits from a single treatment.
- Non-invasive and Cost-Effective: In vivo editing reduces the need for complex, costly procedures like ex vivo cell manipulation, streamlining the treatment process and reducing overall costs.
- Reduced Risk of Immune Reactions: In vivo gene editing lowers the likelihood of immune rejection since edited cells remain within the body, avoiding complications linked to reintroducing externally modified cells.
Examples of In Vivo AAV-CRISPR Editing
1. Liver-Targeted Editing
- Familial Hypercholesterolemia (FH)
- Approach: AAV8 delivers CRISPR-Cas9 to disrupt PCSK9 (a regulator of LDL cholesterol).
- Result: ~50% reduction in PCSK9 levels in mice and non-human primates (NHPs), leading to decreased LDL cholesterol.
- Clinical Relevance: Potential one-time treatment for FH patients.
2. Neurological & Ocular Diseases
- Huntington’s Disease (HD)
- Approach: AAV-CRISPR-Cas9 disrupts mutant HTT in striatal neurons.
- Result: Reduced mutant HTT aggregates and improved motor function in mouse models.
- Leber Congenital Amaurosis 10 (LCA10)
- Approach: AAV5 delivers CRISPR-Cas9 to remove a pathogenic CEP290 intronic mutation.
- Result: Partial vision restoration in animal models.
- Clinical Trial: EDIT-101 (Editas Medicine) – First in-human AAV-CRISPR trial for LCA10.
3. Muscle-Targeted Editing
- Duchenne Muscular Dystrophy (DMD)
- Approach: AAV9-CRISPR excises exon 51 in the DMD gene to restore dystrophin reading frame.
- Result: Functional dystrophin recovery in mice, dogs, and NHPs.
4. Cardiovascular Diseases
- Hypertrophic Cardiomyopathy (HCM)
- Approach: AAV9-CRISPR disrupts mutant MYBPC3 in cardiomyocytes.
- Result: Improved cardiac function in mice.
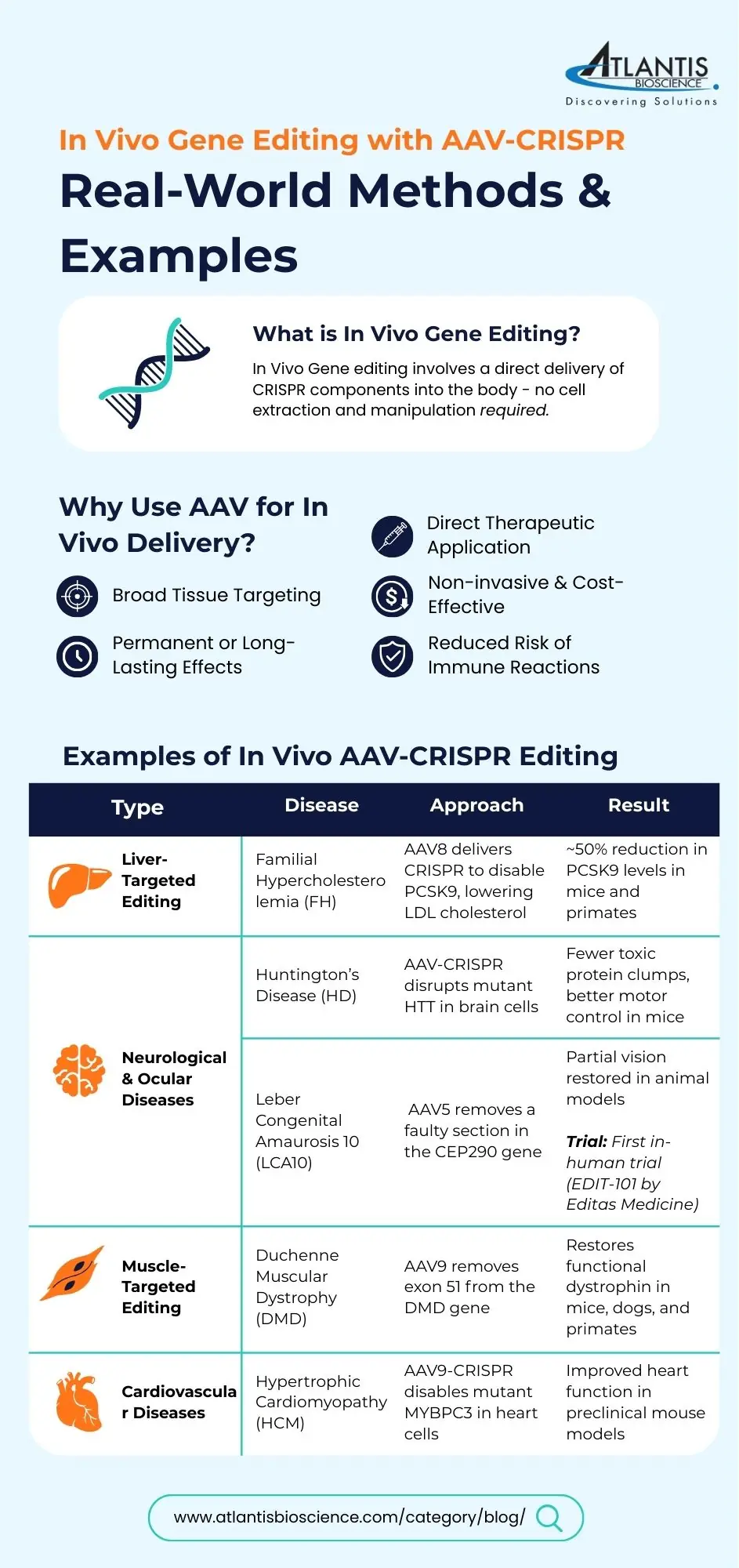
Current Challenges and Future Directions of CRISPR-AAV Therapies
Despite the rapid advances in in vivo AAV-CRISPR gene editing, several technical and translational challenges remain. Overcoming these barriers is critical to fully unlock the therapeutic potential of genome editing for a wide range of diseases. Ongoing innovations in vector design, editing technologies, and manufacturing processes are shaping the future direction of the field, aiming to enhance safety, efficacy, and accessibility for clinical applications.
1. Immune Responses
- Challenge: Many individuals have pre-existing neutralising antibodies (NAbs) against natural AAV serotypes due to prior viral exposure. These antibodies can prevent efficient AAV transduction, limiting the effectiveness of CRISPR gene editing therapies.
- Solutions:
- Capsid engineering: Developing novel AAV capsids that can evade existing immunity while maintaining or improving tissue specificity.
- Immunosuppression strategies: Temporary use of immune modulators during AAV administration is being explored.
- Serotype switching: Administering different AAV serotypes for repeat dosing if necessary.
2. Off-Target Effects
- Challenge: CRISPR-Cas9 can sometimes create unintended double-stranded breaks at sites similar to the target sequence, risking genomic instability, oncogenesis, or other adverse effects.
- Solutions:
- High-fidelity Cas9 variants: Engineered versions such as eSpCas9, HypaCas9, and HiFi Cas9 reduce off-target editing while maintaining high on-target activity.
- Base editors and prime editors: These next-generation editors can perform precise nucleotide changes without inducing double-strand breaks, greatly lowering the risk of off-target mutations.
- Improved gRNA design algorithms: Computational tools help optimise gRNA sequences for specificity.
3. Durability vs. Transience
- Challenge: Permanent genomic modifications are powerful but can be risky if unintended consequences arise. For some therapeutic contexts, it may be preferable to have transient, controllable editing activity.
- Solutions:
- Inducible systems: Drug-regulatable Cas9 systems (e.g., doxycycline-inducible) allow timed control of Cas9 activity.
- Self-inactivating systems: Designs that include self-cleaving elements or self-limiting Cas9 expression reduce long-term exposure to the editing machinery, enhancing safety profiles.
- Transient delivery alternatives: Use of mRNA or protein forms of Cas9 rather than DNA vectors to achieve short-lived but efficient editing.
4. Manufacturing & Scalability
- Challenge: Large-scale production of clinical-grade AAV vectors remains expensive, time-consuming, and technically demanding. Maintaining high titers and purity (low empty capsid ratio) is essential for therapeutic success.
- Solutions:
- Improved production platforms: Triple transfection systems, stable producer cell lines, and baculovirus systems are being optimised for higher yield and scalability.
- Continuous purification technologies: Advances like affinity chromatography (e.g., AVB Sepharose columns) help in more efficient purification of rAAVs.
- Automated and closed-system manufacturing: Reduces contamination risk and increases consistency for large batches.
AAV-mediated CRISPR delivery is a powerful strategy for in vivo genome editing, offering precision, tissue specificity, and long-term effects. While challenges like packaging limits and immune responses persist, innovations in miniaturised editors, split systems, and regulatable Cas9 are pushing the field forward.
Clinical trials (e.g., EDIT-101 for LCA10) demonstrate the real-world potential of AAV-CRISPR therapies. As research advances, we can expect more breakthroughs in treating genetic disorders directly within patients.
PackGene’s AAV and Off-the-Shelf CRISPR/Cas9 rAAV Solutions
PackGene Biotech has emerged as a leader in AAV vector design, manufacturing, and CRISPR-related therapeutic development. They provide both custom AAV services and ready-to-use (off-the-shelf) CRISPR/Cas9 rAAV products that significantly accelerate in vivo gene editing research.
PackGene AAV Capabilities:
- High-yield manufacturing: PackGene uses proprietary technology platforms (e.g., optimised cell lines and enhanced RC and helper plasmids) to achieve superior vector yields (up to 1E+16 GC) with high purity and low empty capsid ratios.
- Wide serotype selection: Includes natural serotypes (like AAV2, AAV8, AAV9) and engineered variants for tissue-specific targeting.
- Regulatory-grade production: GMP-compliant manufacturing supports preclinical through clinical applications.
Off-the-Shelf CRISPR/Cas9 rAAV Products:
- Cas9 is expressed under EFS, miniCMV, CMV, or various tissue-specific promoters.
- Available in multiple serotypes, including AAV2, AAV8, AAV9, and AAV-PHP.eB.
- In-stock and ready-to-ship within one business day, ideal for fast-paced or time-sensitive projects.
- Also offered in High-Fidelity Cas9 variants to minimise off-target effects, as well as Cpf1 versions for alternative genome editing.
Ready to Accelerate Your CRISPR Research?
PackGene’s off-the-shelf CRISPR/Cas9 rAAV products are designed to deliver high-quality, reliable results — with rapid turnaround times to keep your projects moving forward. Whether you need broad expression or tissue-specific targeting, our pre-made AAVs are ready to support your in vivo genome editing needs.
Explore our product catalog today or contact our team to discuss how we can help power your next breakthrough!
References
- Amoasii L, Hildyard JCW, Li H, Sanchez-Ortiz E, Mireault A, Caballero D, Harron R, Stathopoulou TR, Massey C, Shelton JM, Bassel-Duby R, Piercy RJ, Olson EN. Gene editing restores dystrophin expression in a canine model of Duchenne muscular dystrophy. Science. 2018 Oct 5;362(6410):86-91. doi: 10.1126/science.aau1549. Epub 2018 Aug 30.
- Greer-Short, A., Greenwood, A., Leon, E.C. et al. AAV9-mediated MYBPC3 gene therapy with optimized expression cassette enhances cardiac function and survival in MYBPC3 cardiomyopathy models. Nat Commun 16, 2196 (2025). https://doi.org/10.1038/s41467-025-57481-7
- Lau CH, Suh Y. In vivo genome editing in animals using AAV-CRISPR system: applications to translational research of human disease. F1000Res. 2017 Dec 20;6:2153. doi: 10.12688/f1000research.11243.1.
- Maeder ML, Stefanidakis M, Wilson CJ, Baral R, Barrera LA, Bounoutas GS, Bumcrot D, Chao H, Ciulla DM, DaSilva JA, Dass A, Dhanapal V, Fennell TJ, Friedland AE, Giannoukos G, Gloskowski SW, Glucksmann A, Gotta GM, Jayaram H, Haskett SJ, Hopkins B, Horng JE, Joshi S, Marco E, Mepani R, Reyon D, Ta T, Tabbaa DG, Samuelsson SJ, Shen S, Skor MN, Stetkiewicz P, Wang T, Yudkoff C, Myer VE, Albright CF, Jiang H. Development of a gene-editing approach to restore vision loss in Leber congenital amaurosis type 10. Nat Med. 2019 Feb;25(2):229-233. doi: 10.1038/s41591-018-0327-9. Epub 2019 Jan 21.
- Nelson CE, Hakim CH, Ousterout DG, Thakore PI, Moreb EA, Castellanos Rivera RM, Madhavan S, Pan X, Ran FA, Yan WX, Asokan A, Zhang F, Duan D, Gersbach CA. In vivo genome editing improves muscle function in a mouse model of Duchenne muscular dystrophy. Science. 2016 Jan 22;351(6271):403-7. doi: 10.1126/science.aad5143. Epub 2015 Dec 31.
- Rothgangl T, Dennis MK, Lin PJC, Oka R, Witzigmann D, Villiger L, Qi W, Hruzova M, Kissling L, Lenggenhager D, Borrelli C, Egli S, Frey N, Bakker N, Walker JA 2nd, Kadina AP, Victorov DV, Pacesa M, Kreutzer S, Kontarakis Z, Moor A, Jinek M, Weissman D, Stoffel M, van Boxtel R, Holden K, Pardi N, Thöny B, Häberle J, Tam YK, Semple SC, Schwank G. In vivo adenine base editing of PCSK9 in macaques reduces LDL cholesterol levels. Nat Biotechnol. 2021 Aug;39(8):949-957. doi: 10.1038/s41587-021-00933-4. Epub 2021 May 19.
- Sconocchia T, Foßelteder J, Köhnke T, Majeti R, Reinisch A. Engineering Oncogenic Heterozygous Gain-of-Function Mutations in Human Hematopoietic Stem and Progenitor Cells. J Vis Exp. 2023 Mar 10;(193). doi: 10.3791/64558.
- Wang D, Zhang F, Gao G. CRISPR-Based Therapeutic Genome Editing: Strategies and In Vivo Delivery by AAV Vectors. Cell. 2020 Apr 2;181(1):136-150. doi: 10.1016/j.cell.2020.03.023.
- Xin, C., Yin, J., Yuan, S. et al. Comprehensive assessment of miniature CRISPR-Cas12f nucleases for gene disruption. Nat Commun 13, 5623 (2022). https://doi.org/10.1038/s41467-022-33346-1
- Yang S, Chang R, Yang H, Zhao T, Hong Y, Kong HE, Sun X, Qin Z, Jin P, Li S, Li XJ. CRISPR/Cas9-mediated gene editing ameliorates neurotoxicity in mouse model of Huntington’s disease. J Clin Invest. 2017 Jun 30;127(7):2719-2724. doi: 10.1172/JCI92087. Epub 2017 Jun 19